- Home
- Member Resources
- Articles
- Advancements in Transfusion Medicine: Towards the Era of Universal Blood
The field of transfusion medicine (TM) plays a crucial role in healthcare with approximately 16 million blood components transfused annually in the US, saving lives in emergencies, surgeries, cancer treatments, and various medical conditions.1 TM has always been on the forefront of precision medicine.2 Karl Landsteiner’s discovery of ABO blood groups and Rh factor in the early 20th century led to the provision of matched blood products to patients to prevent hemolytic complications and enhance safety.3 Today, serologic and molecular techniques, along with laboratory information systems and electronic health records, contribute to precise blood product management and personalized transfusion therapies, particularly benefiting complex patients with sickle cell disease, thalassemia, and other diseases requiring chronic transfusion support.4,5 As precision medicine evolves, TM continues to revolutionize patient care by optimizing transfusion strategies and minimizing risks associated with incompatible blood products.
Nevertheless, the field of TM grapples with a fragile blood supply, a situation exacerbated by the COVID-19 pandemic. Thousands of blood drives were canceled in the early weeks of the pandemic, resulting in a significant deficit of about 150,000 red blood cell (RBC) units according to the American Red Cross (ARC). ARC also declared its first blood crisis during the Omicron surge. Resilient blood centers implemented operational changes and intensified donor recruitment efforts to stabilize the situation.6,7 However, the aftershocks of the pandemic disruptions persist, compounded by workforce and supply shortages.8 Moreover, a dwindling and aging donor pool poses an ongoing challenge.9 Blood centers are actively urging healthy donors to contribute, bearing in mind that only 3% of eligible Americans donate blood. However, they continue to struggle with meeting the demand, leading to recurring temporal and regional blood shortages in the new normal.
Most clinically significant shortages are seen in the availability of universal blood, ie, type O RBC. Type O blood is compatible with all blood types and is needed during urgent/emergent surgeries, trauma, and other situations where blood bank testing cannot be completed, and blood products are needed as an immediate lifesaving measure.10 The presence of ABO antigens on RBC surfaces requires that careful blood-typing must be carried out prior to transfusion to avoid adverse and sometimes fatal hemolytic transfusion reactions.11 The promise of developing universal blood could eliminate the barrier of matching ABO antigens for blood transfusions, which is time-consuming and limits inventory. Researchers are investigating several approaches, such as enzymatic treatments of RBCs, the generation of RBCs from human induced pluripotent stem cells, and the development of artificial oxygen carriers, all with the goal of advancing universal blood. These efforts aim to address challenges like blood shortages, alloimmunization, and improve the overall success and safety of transfusions.
Enzymatically Treated RBC
Glycoprotein antigens on the extracellular surface of the RBC membrane determine an individual's blood group. The H-antigen with the fucosyl galactose end chain is present on type O blood and is the base structure of the ABO blood group system. Other blood types differ from type O in the presence of an additional sugar antigen, α- N-acetyl galactose for type
A, α-galactose for type B, or both for type AB, on the core of the nonimmunogenic H-antigen (Figure 1). This basic biochemistry concept forms the basis for developing universal type O blood by enzyme treatment. In the 1980s, Goldstein demonstrated type B to type O conversion using α-galactosidase enzymes.12,13 Units prepared from this method, in follow-up studies in the early 1990s, were transfused to healthy volunteers with no ill effects and normal RBC circulatory survival times.14,15 A larger, phase I clinical trial carried out in 2000 also showed similar results.16 With the goal of large-scale production of enzyme-treated universal RBC, the focus was turned on treating type A blood (40% of donor population). α-N-acetyl-galactosaminidase allowed for the conversion of A-antigen to H-antigen (type-O RBC). Phase I trials conducted in 2005 showed that small volumes of enzyme-treated type A RBC can be reinfused into the original donor with no ill effects.17 Despite the early success of creating universal RBC without adverse effects, the technology has not yet moved into clinical practice. Major hurdles include the large amount of enzymes needed for converting RBC types and the low levels of agglutination observed due to residual type A/B antigens left after treatment. Further studies are needed to determine the clinical significance of residual antigens and subsequent agglutination. Newer methods that require lower enzyme doses and a better understanding of observed agglutination issues will help move enzyme-treated universal blood further into clinical practice.18
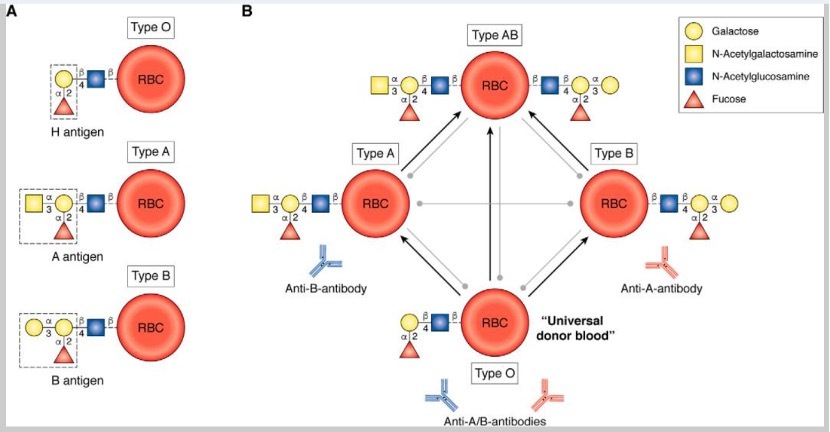
RBC Generated from Human Induced Pluripotent Stem Cells
Human induced pluripotent stem cells (iPSCs) hold great promise in the quest for developing universal blood.19 Unlike other sources of progenitors, such as cord blood, bone marrow, and peripheral blood stem cells, iPSCs have the potential for large-scale generation of transfusable RBCs. iPSCs are created from healthy donor somatic cells, cultured in chemically defined growth media to reach optimal concentration, and then exposed to specific factors that induce their maturation into oxygen-carrying RBCs (Figure 2). The use of iPSCs can address chronic blood supply challenges, alloimmunization (the development of antibodies against non-ABO antigens), which is a significant obstacle for patients with conditions like thalassemia or sickle-cell disease, and increase the availability of blood for patients with rare phenotypes. The first successful in-vitro differentiation of iPSCs into functional RBCs was reported in 2006.20 A murine model demonstrated that iPSC-derived RBCs circulated similarly to normal human RBCs, homed in the bone marrow of immunodeficient mice, and retained the ability to differentiate into enucleated erythrocytes and enter the peripheral blood.21
Recent preclinical studies have shown potential for large-scale production of iPSC-derived RBCs.22 CRISPR gene editing has been used in conjunction with iPSCs to create specialized cells that can generate rare donor cells or produce a source of universal RBCs with specific phenotypes.23
Significant progress has been made in selecting the initial cell type, reprogramming methods, ensuring the clinical-grade safety of iPSCs, optimizing erythrocyte differentiation, and implementing GMP conditions for industrial-scale production. However, it is important to note that iPSC-derived RBCs are still awaiting clinical trials to demonstrate their readiness for widespread use.24
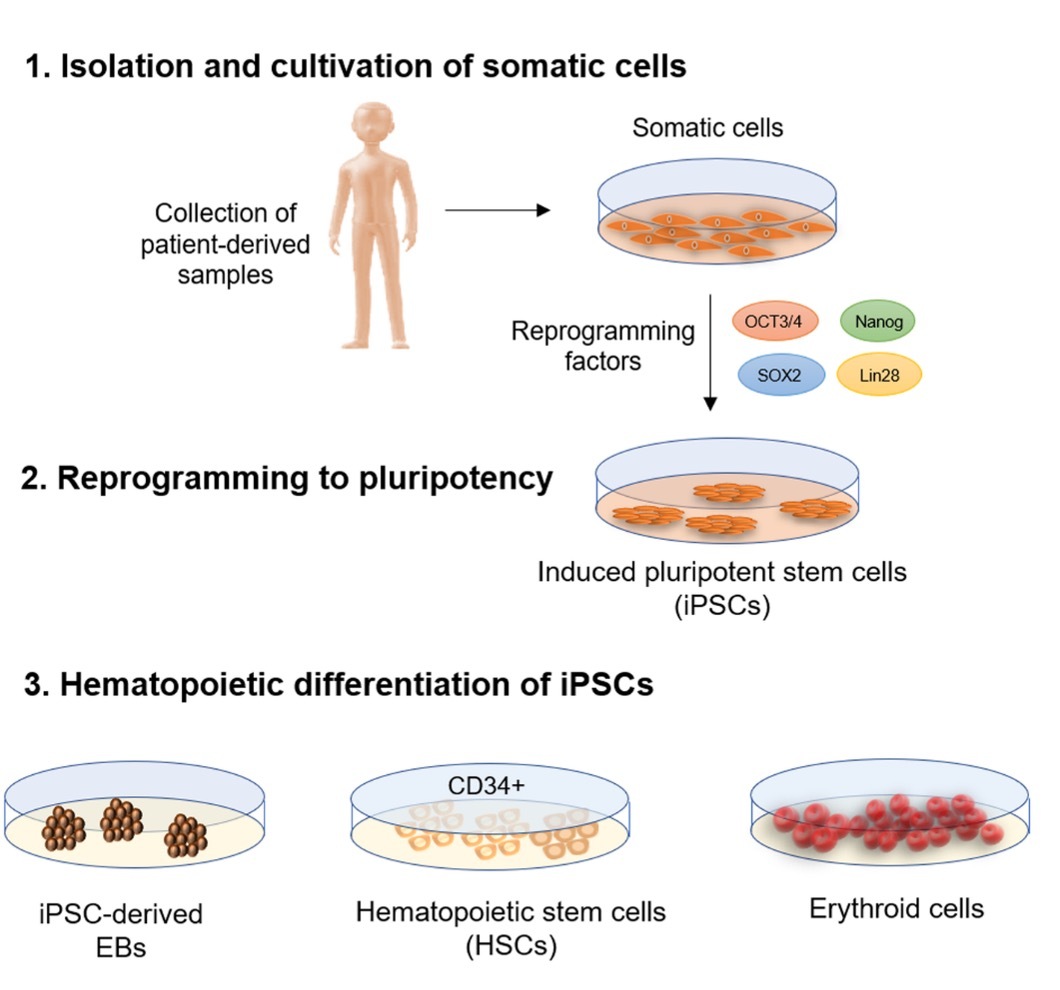
Artificial Oxygen Carriers
Hemoglobin within RBCs consists of four globin protein chains, two α and two β chains in adults, each attached to a heme group containing an iron ion. This iron ion plays a crucial role in oxygen transport by binding and releasing oxygen molecules.26 Artificial oxygen carriers (AOCs) serve as an alternative option for resuscitation when blood transfusion is not an option due to challenges in terms of availability, type matching, portability, and shelf-life. These carriers can deliver oxygen and maintain volume effectively. Hemoglobin-based oxygen carriers (HBOCs) are either semisynthetic or synthetic substitutes for RBCs, where cell-free hemoglobin is stabilized through methods such as cross-linking, conjugation, polymerization, or encapsulation.27 Perfluorocarbon-based oxygen carriers (PFOCs) are chemical compounds composed solely of carbon and fluorine atoms and possess the capacity for oxygen transport. There have been a number of clinical trials of AOCs, but the results have been mixed.
After undergoing numerous phases of clinical trials (phases I-III), one of the HBOCs, Hemopure, gained approval as an oxygen carrier in South Africa in 2001 and in Russia in 2012. However, the Food and Drug Administration (FDA) has not granted approval for it, making it available solely under the FDA Expanded Use protocol, primarily for compassionate use.28 Another HBOC, Sanguinate, completed a Phase II trial in 2017 to assess its safety and efficacy in sickle cell patients experiencing vaso-occlusive crises. The results of this trial are still pending. Several phase I-III trials involving other HBOCs were terminated early due to significant safety concerns associated with these products.29 These concerns include the risk of hemolysis, oxidative damage, renal toxicity, nitric oxide (NO) NO scavenging, and hypertension. In contrast, PFOCs offer advantages such as higher oxygen-carrying capacity, chemical stability, longer shelf life, and a lack of issues related to hemoglobin toxicity or NO-scavenging. However, no PFOCs have received FDA approval for clinical use thus far. Many PFOCs are currently in the process of completing phase I-III clinical trials to demonstrate their potential as therapeutic options.30 AOCs continue to be studied and evaluated for their effectiveness, safety, and long-term outcomes. They aim to establish themselves as viable alternatives or universal blood options in clinical scenarios such as traumatic exsanguination, hyperhemolysis, and autoimmune hemolysis that do not respond to traditional transfusions.
In conclusion, the pursuit of universal blood through enzymatically treated RBCs, RBCs derived from human induced pluripotent stem cells, and artificial oxygen carriers represents a promising frontier in transfusion medicine. These approaches hold the potential to mitigate blood shortages, enhance transfusion safety, and transform patient care by removing the constraints of blood type compatibility. As shown in Table 1, which compares these three innovative strategies, each method presents unique advantages and challenges. While challenges and questions remain, ongoing research and clinical trials are crucial to harnessing the full potential of these innovations. As these strategies advance, they offer hope for a more resilient and efficient transfusion system, ushering in a new era of healthcare where life-saving blood products are more universally accessible and adaptable to the needs of diverse patients.
Table 1: Comparison of Approaches for Universal Blood
iPSC-derived RBCs |
Enzyme-treated RBCs |
Artificial oxygen carriers |
|
Source |
Human pluripotent stem cells |
Human RBCs |
Synthetic compounds |
Oxygen-carrying capacity |
Similar to natural RBCs |
Similar to untreated RBCs |
Exhibit a strong affinity for oxygen binding |
Production cost |
High |
High |
Low |
Production time |
Long |
Short |
Short |
Shelf-life |
Limited by the lifespan of natural RBCs |
Similar lifespan to untreated RBCs |
Longer shelf life |
Safety |
Not yet fully established |
Some safety concerns from enzymes used and agglutination due to residual antigens. |
Some safety concerns due to hemolysis, oxidative damage, and nitric oxide scavenging. |
Clinical application |
Long-term blood support, rare blood types |
To reduce the adverse effects associated with transfusions |
Acute blood transfusions, trauma, surgery |
References
- American Red Cross. Blood needs and the blood supply. https://www.redcrossblood.org/donate-blood/how-to-donate/how-blood-donations-help/blood-needs-blood-supply.html
- Klein HG, Flegel WA, Natanson C. Red blood cell transfusion: precision vs imprecision medicine. JAMA. 2015;314(15):1557-1558. doi: 10.1001/jama.2015.10890.
- Durand JK, Willis MS. Karl Landsteiner, MD: transfusion medicine. Lab Med. 2010:53-55.
- Sapatnekar S, Figueroa PI. How do we use molecular red blood cell antigen typing to supplement pretransfusion testing? Transfusion. 2014;54(6):1452-1458. doi: 10.1111/trf.12623.
- Crispin P, Akers C, Brown K, et al. A review of electronic medical records and safe transfusion practice for guideline development. Vox Sang. 2022;117(6):761-768. doi: 10.1111/vox.13254.
- Mathur G. Patient blood management strategies during Coronavirus disease 2019 pandemic. Open J Cardiol Heart Dis. 2022. doi:10.31031/OJCHD.2022.04.000576.
- American Red Cross. Blood donors needed now as Omicron intensifies. https://www.redcross.org/about-us/news-and-events/press-release/2022/blood-donors-needed-now-as-omicron-intensifies.html
- AABB. Workforce issues compound blood collectors' challenges. https://www.aabb.org/news-resources/news/article/2022/04/13/workforce-issues-compound-blood-collectors-challenges
- Tufts Now. What is Causing the National Blood Shortage? https://now.tufts.edu/2022/03/04/what-causing-national-blood-shortage
- Barty RL, Pai M, Liu Y, et al . Group O RBCs: where is universal donor blood being used. Vox Sang. 2017;112(4):336-342. doi: 10.1111/vox.12492
- Panch SR, Montemayor C, Klein HG. Hemolytic transfusion reactions. N Engl J Med. 2019;381(2):150-162. doi: 10.1056/NEJMra1802338.2
- Lenny L, Goldstein J. Enzymatic removal of blood group B antigen from gibbon erythrocytes. Transfusion. 1980:618.
- Goldstein J, Siviglia G, Hurst R, et al. Group B erythrocytes enzymatically converted to group O survive normally in A, B, and O individuals. Science. 1982;215(4529):168-170. doi: 10.1126/science.6274021.
- Lenny LL, Hurst R, Goldstein J, Benjamin LJ, Jones RL. Single-unit transfusions of RBC enzymatically converted from group B to group O to A and O normal volunteers. Blood. 1991;77(6):1383-1388..
- Lenny LL, Hurst R, Zhu A, Goldstein J., Galbraith RA. Multiple‐unit and second transfusions of red cells enzymatically converted from group B to group O: report on the end of phase 1 trials. Transfusion. 1995;35(11):899-902. doi:10.1046/j.1537-2995.
- Kruskall MS, AuBuchon JP, Anthony KY, et al. Transfusion to blood group A and O patients of group B RBCs that have been enzymatically converted to group O. Transfusion. 2000;40(11):1290-1298. doi:10.1046/j.1537-2995.2000.40111290.x.
- Olsson ML, Clausen H. Modifying the red cell surface: towards an ABO‐universal blood supply. Br J Haematol. 2008;140(1):3-12. doi:10.1111/j.1365-2141.2007.06839.x.
- Rahfeld Pr, Withers SG. Toward universal donor blood: Enzymatic conversion of A and B to O type. J Biol Chem. 2020;295(2):325-334. doi:10.1074/jbc.REV119.008164. Reused under license CC-BY-4.0
- Seifinejad A , Taei A, Totonchi M, et al. Generation of human induced pluripotent stem cells from a Bombay individual: moving towards “universal-donor” red blood cells. Biochem Biophys Res Commun. 2010;391(1):329-334. doi:10.1016/j.bbrc.2009.11.058.
- Mazurier C, Douay L, Lapillonne H. Red blood cells from induced pluripotent stem cells: hurdles and developments. Curr Opin Hematol. 2011;18(4):249-53. doi: 10.1097/MOH.0b013e3283476129.
- Deng J, , Lancelot M, Jajosky R, et al. Erythropoietic properties of human induced pluripotent stem cells‐derived red blood cells in immunodeficient mice. Am J Hematol. 2022;97(2):194-202. doi:10.1002/ajh.26410.
- Claessen M, Varga E, Heshusius S, et al. Large scale culture and differentiation of erythroblasts from PBMC and iPSC. Blood 2018:2319.
- Hawksworth J, Satchwell TJ, Meinders M, et al. Enhancement of red blood cell transfusion compatibility using CRISPR‐mediated erythroblast gene editing. EMBO Mol Med. 2018;10(6):e8454. doi:10.15252/emmm.201708454.
- Lee SJ, Jung C, Oh JE, et al. Generation of red blood cells from human pluripotent stem cells—an update. Cells. 2023;12(11):1554. doi:10.3390/cells12111554.
- Ebrahimi M, Forouzesh M, Raoufi S, Ramazii M, Ghaedrahmati F, Farzaneh M. Differentiation of human induced pluripotent stem cells into erythroid cells. Stem Cell Res Ther. 2020;11(1):483. doi:10.1186/s13287-020-01998-9. Reused under license CC-BY-4.0.
- Jensen, FB, Fago A, Weber RE. Hemoglobin structure and function. Fish Physiol. 1998: 1-40.
- Gupta, AS. Hemoglobin-based oxygen carriers: current state-of-the-art and novel molecules. Shock. 52(1S Suppl 1): 70-83. doi: 10.1097/SHK.0000000000001009.
- Jahr JS, Guinn NR, Lowery DR, Shore-Lesserson L, Shander A. Blood substitutes and oxygen therapeutics: a review. Anesth Analg. 2021;132(1):119-129. doi: 10.1213/ANE.0000000000003957.
- Mohanto, N, Park YJ, Jee JP. Current perspectives of artificial oxygen carriers as red blood cell substitutes: a review of old to cutting-edge technologies using in vitro and in vivo assessments. J Pharm Investig. 2023;53(1):153-190. doi: 10.1007/s40005-022-00590-y.
- Mohanto, N, Park YJ, Jee JP. Current perspectives of artificial oxygen carriers as red blood cell substitutes: a review of old to cutting-edge technologies using in vitro and in vivo assessments. J Pharm Investig. 2023;53(1):153-190. doi:10.1007/s40005-022-00590-y.
Roksolana Demianets, MD, is a second-year pathology resident at the University of California, Irvine. Dr. Demianets earned her medical degree from the Ivano-Frankivsk National Medical University, Ukraine. Her research interests focus on the effectiveness and safety of transfusion practices, and rare hematologic entities, including plasmablastic lymphoma and hemophagocytic lymphohistiocytosis.
Sumayya Aslam, MD, is a PGY 2 resident at the University of California, Irvine. She earned her medical degree at Dow Medical College in Pakistan. She has a research interest in hematopathology and gastropathology. She will be applying for a hematopathology fellowship for the 2026-2027 academic year and hopes to pursue a career in hematopathology in an academic setting.
Gagan Mathur, MD, MBA, CPE, FCAP, is a board-certified transfusion medicine/blood banking physician and serves as the medical director of transfusion medicine at the University of California, Irvine (UCI). Dr. Mathur holds the academic title of associate professor of clinical pathology in the Department of Pathology & Laboratory Medicine at the UCI School of Medicine. He pursued his master of business administration (MBA) from the University of Iowa Tippie School of Management, along with his clinical training in clinical pathology at the University of Iowa Hospitals & Clinics. Dr. Mathur earned the title of Certified Physician Executive from American Association for Physician Leadership. Dr. Mathur’s areas of clinical interest and expertise include patient blood management, therapeutic apheresis, and cellular therapies. Along with his clinical responsibilities, Dr. Mathur is enthusiastically involved in clinical research and teaching to accomplish his academic commissions. Utilizing his interest and training in business management, informatics, and healthcare administration, Dr. Mathur strives to provide effective and efficient patient care.